Enhancing the observational method through digital innovation
DAARWIN[1] is a web platform that refines geotechnical design models using data collected from site. It compares the design prediction against the measured response to enable a more accurate understanding and future analysis of the ground and ground-structure interaction behaviours. This paper describes how this was trialled on HS2.
The primary aim of the trial was to test the DAARWIN technology, which is the first ground engineering software to use machine learning algorithms to link design models with instrumentation and monitoring data, accelerating back-analysis and progressive modification cycles to enhance the implementation of the Observational Method. DAARWIN is cloud-based, enabling very large volumes of data to be managed and easily accessed. This supports the use of the Observational Method on much larger scale applications than was possible by conventional methods. The platform also supports the creation of a Digital Twin for underground infrastructure. The Observational Method can deliver major savings in construction programme whilst enhancing safety as well as contributing to meeting sustainability goals, such as reducing CO2 emission through leaner design and better construction control.
The trial revealed opportunities to eliminate heavy and constricting temporary works for excavation projects using similar construction methodology located in similar geological conditions, provided the Observational Method is used effectively. On projects the size of HS2, with a great length of retained excavations, the potential efficiency gains are significant.
Ground risks resulting in construction cost and time overruns
The construction industry has a poor track-record on delivering projects to budget and on time. Nine in ten projects experience cost overruns and 40% of the cases directly relate to a lack of understanding of ground behaviour. Risk of failure during construction and operation is also linked to geotechnical uncertainties, and can cause service closures, increased maintenance costs, and may endanger life and property.
Climate change (Horizon Europe: Strategic Plan 2021–2024[2] & Eurocode7[3]) exacerbates the need for leaner design and construction and reduced carbon expenditure. Extreme weather events, leading to greater variance in groundwater conditions affects ground stability and behaviour contributing to geotechnical uncertainty. Traditionally, geotechnical uncertainty is managed in design by increasing safety factors and adopting more conservative assessments of strength and stiffness and during construction through inspection and monitoring. This often results in over-conservative design and wasteful construction practices. A better definition of geotechnical uncertainties allows for leaner permanent and temporary designs, potential to reduce construction cost, duration, and carbon.
After the first and very successful experience testing DAARWIN on HS2 within the framework of an Innovation DISCOVERY Project[4] in 2020, HS2’s Innovation team decided to trial DAARWIN further across more parts of HS2 within the framework of an Innovation STRATEGIC Project. One of the parts of HS2 where it was decided to undertake the trial was on the Phase 1 – N1/N2 Main Works Civils Contract at Bromford Tunnel East Portal (BTEP) in collaboration with the Integrated Project Team Area North (IPTAN) being the design-and-build contractor, Balfour Beatty Vinci Joint Venture, their designer, Mott MacDonald Systra Design Joint Venture, and their Category 3 Checker, ARUP.
Using the observational method to overcome cost and time overruns in construction
Introduction to the Observational Method
The Observational Method (OM) is essentially an integration of design and construction control, linking design to observed performance during construction. Unlike a traditional design, it provides a “feedback loop” between design and construction through close monitoring of ground and structure behaviour, and construction control to enable pre-planned design modifications to be implemented progressively during construction (Powderham, 2002[5]; Powderham and O’Brien, 2021[6]). It has a well-established technical basis, for example CIRIA 185 by Nicholson et al (1999)[7].
Since the 1980s, Mott MacDonald’s engineers have helped pioneer the development of the OM to optimise the delivery of major projects including Limehouse Link, the Heathrow Express rail tunnel, Heathrow Airport Terminal 5, Northern Line Extension, and the Elizabeth Line’s Moorgate Shaft (Powderham and O’Brien, 2021[6]; Liew et al, 2016[8]; O’Brien and Liew, 2018[9]). They showed that the OM, when used effectively, can deliver major cost, time, and carbon savings; as well as enhanced safety by focusing on good communication, effective collaboration of the design and construction team, clearer procedures and control during construction (O’Brien et al, 2022[10]). On projects the size of HS2, with a great length of retained excavations and earthworks, the potential efficiency gains are significant.
Despite the many practical benefits of the OM, it is still significantly under-used. Apart from inappropriate contracts and cultural issues which have been described in detail by O’Brien et al (2022)[10], there are many misconceptions about OM which can result in inadequate stakeholder support. It is often misconstrued as a method that might increase programme uncertainties or risks in projects, or it may only be used when a project has already encountered a problem such as being behind schedule. Hence, OM is often perceived as a reactive rather than a proactive approach. Therefore, gaining a “buy-in” from stakeholders can often be a major challenge. The ‘art of achieving agreement’ to implement the OM is discussed by Powderham and O’Brien (2021)[6]. In practice, experienced OM practitioners need to persevere in explaining OM to key stakeholders. Hence, considerable effort is required to create a more effective and collaborative working environment. A “single team” mentality with close teamwork and mutual trust is essential for OM to be implemented.
DAARWIN platform
DAARWIN is a cloud-based platform based on machine learning algorithms that gathers data, creates connections between design and construction, visualizes construction performance against design analysis, enables ‘real-time’ back-analysis to enable modifications to design and construction to be made based on actual performance, and better manage risk during underground construction (De Santos, 2015[11]).
DAARWIN refines geotechnical design models using data collected from site. It compares the design prediction against the measured response to enable a more accurate understanding and future analysis of the ground and ground-structure interaction behaviours. The resulting back-analysed parameters can be used to modify the existing design and construction sequence through the application of the OM to make them more sustainable, efficient and safer.
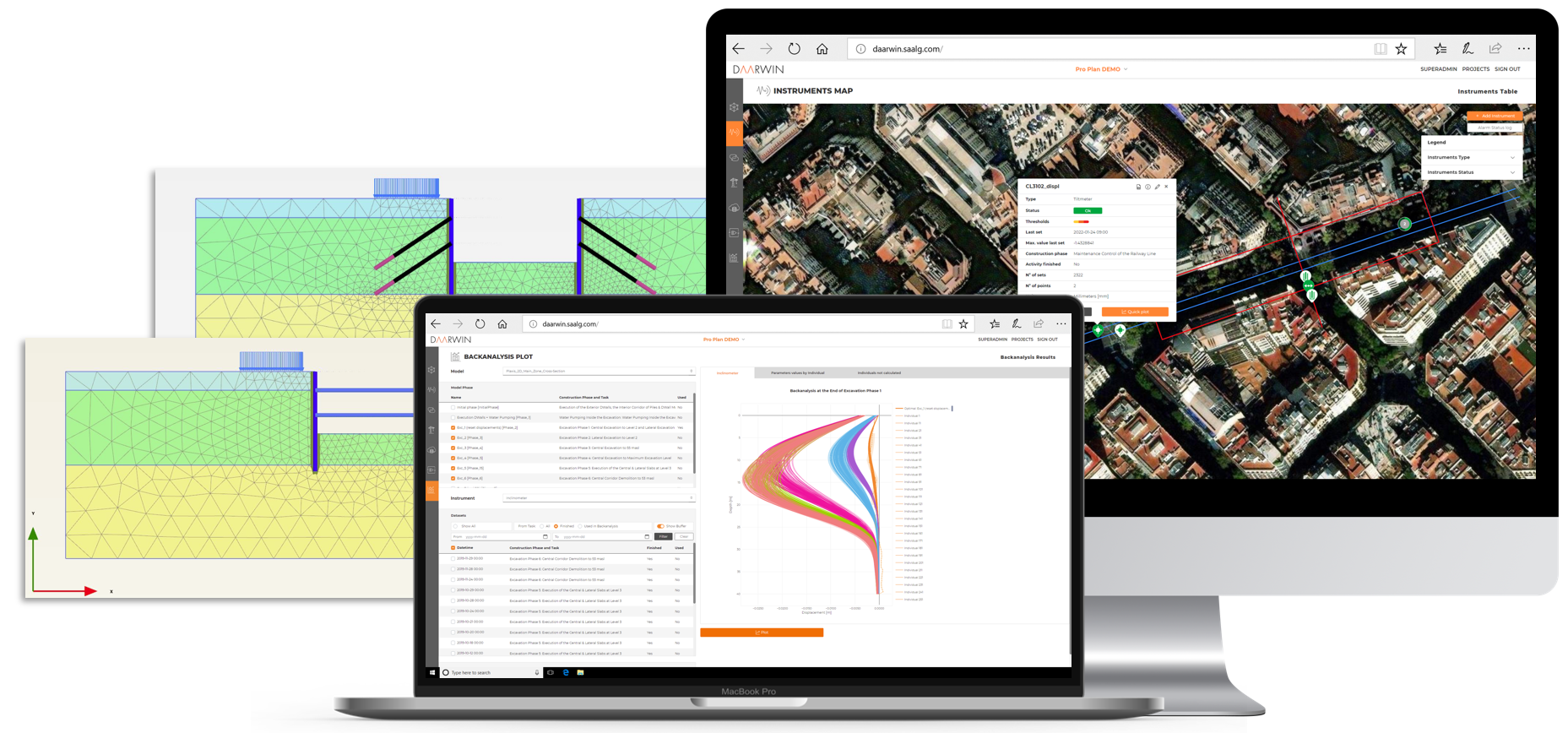
Despite the significant practical benefits of carrying out back-analysis, it is not common practice due to many technical and practical challenges. A wide range of skills are required for any engineers carrying out back-analysis, e.g. understanding both structural and geotechnical behaviour, construction processes, advanced analysis, interpretation of instrumentation and monitoring data. There are also practical and logistical challenges in handling and managing large volumes of data. Back-analysis is the process where model parameters are changed until an improved match with monitoring data is achieved. Once validated, the model can be extrapolated to make forward predictions of ground and structural behaviour under different conditions. Back-analysis is traditionally performed manually following trial-and-error. With the number of parameters in ground models increasing (especially for more advanced constitutive models), back-analysis typically takes three to six weeks. By this time, construction has frequently progressed, or expensive mitigating measures have been implemented without benefitting from the experience acquired through back-analysis. Therefore, accelerating back-analysis to near real-time has the potential to transform geotechnical practice, and significantly enhances the power of the OM. DAARWIN makes it possible to execute back analysis regularly, in near real-time. Because DAARWIN is also a data management platform it can also be a valuable repository of geotechnical information which, if used wisely, can be used to create leaner future designs.
How DAARWIN works
- Upload the project information into the platform. Numerical models, construction progress monitoring data, images and historical information can be uploaded.
- Analyse multiple design options together with different ground parameter scenarios (from pessimistic, most probable to optimistic) to determine the most optimal design option and the most influential geotechnical parameters.
- Compare the design with the monitoring data to prove that the construction is performing according to design (i.e., confirm the design and construction works are safe and verify the approach).
- Calibrate the numerical models to predict the real ground and ground – structure behaviour, and implement the Observational Method by Progressive Modification (OMPM), to minimise construction material (less CO2 emissions), delays and accidents in a project.
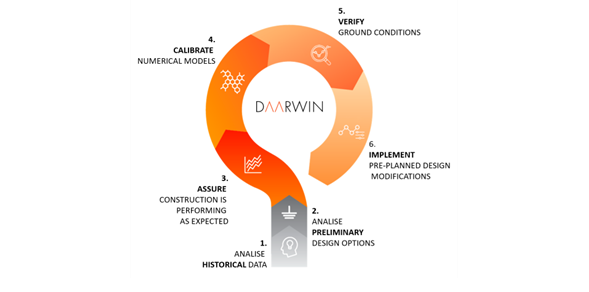
The benefits of being connected
DAARWIN enables the connection between all the stakeholders of a project, which for this trial on HS2 included the client (HS2), their technical assurance team (TAA), the design-and-build contractor and their designer, and the independent checking team, to make wiser decisions to plan, deliver and manage more sustainable, efficient, and safer projects.
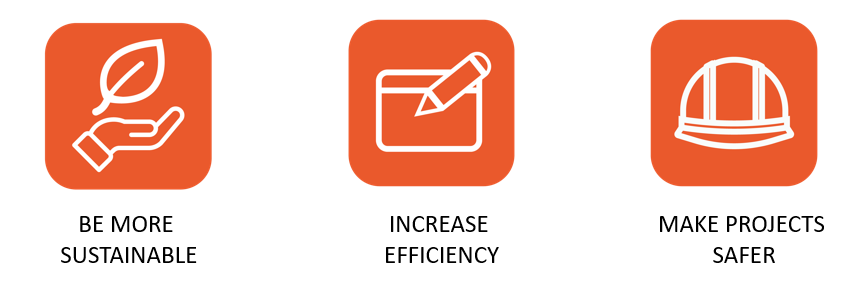
- Reduce over-dimensioning to minimise construction material consumption and CO2 emissions. Currently the construction industry is responsible for more than 10% of the global CO2 emissions and this must change. Uncertainty related to the ground and ground risks contributes significantly to the potential over-dimensioning of geotechnical structures. By understanding the behaviour of the ground and ground-structure interaction in near real time, enables the team to implement progressive modification to reduce this over-dimensioning.
- Digitalise the entire lifecycle of a project to make data-driven decisions easier and faster. Efficiency and productivity in construction has grown an average of 1% a year over the past two decades, compared with the growth of 2.8% for the total economy. As presented in the Gemini Paper, digitalisation, and especially digital twins, are tools to turn data into insights that enable improved decision and provide better outcomes (see Figure 4).
- Detect instabilities in advance and prove construction is performing according to the design (i.e., minimise the potential for unsafe construction) and reduce ground risks.
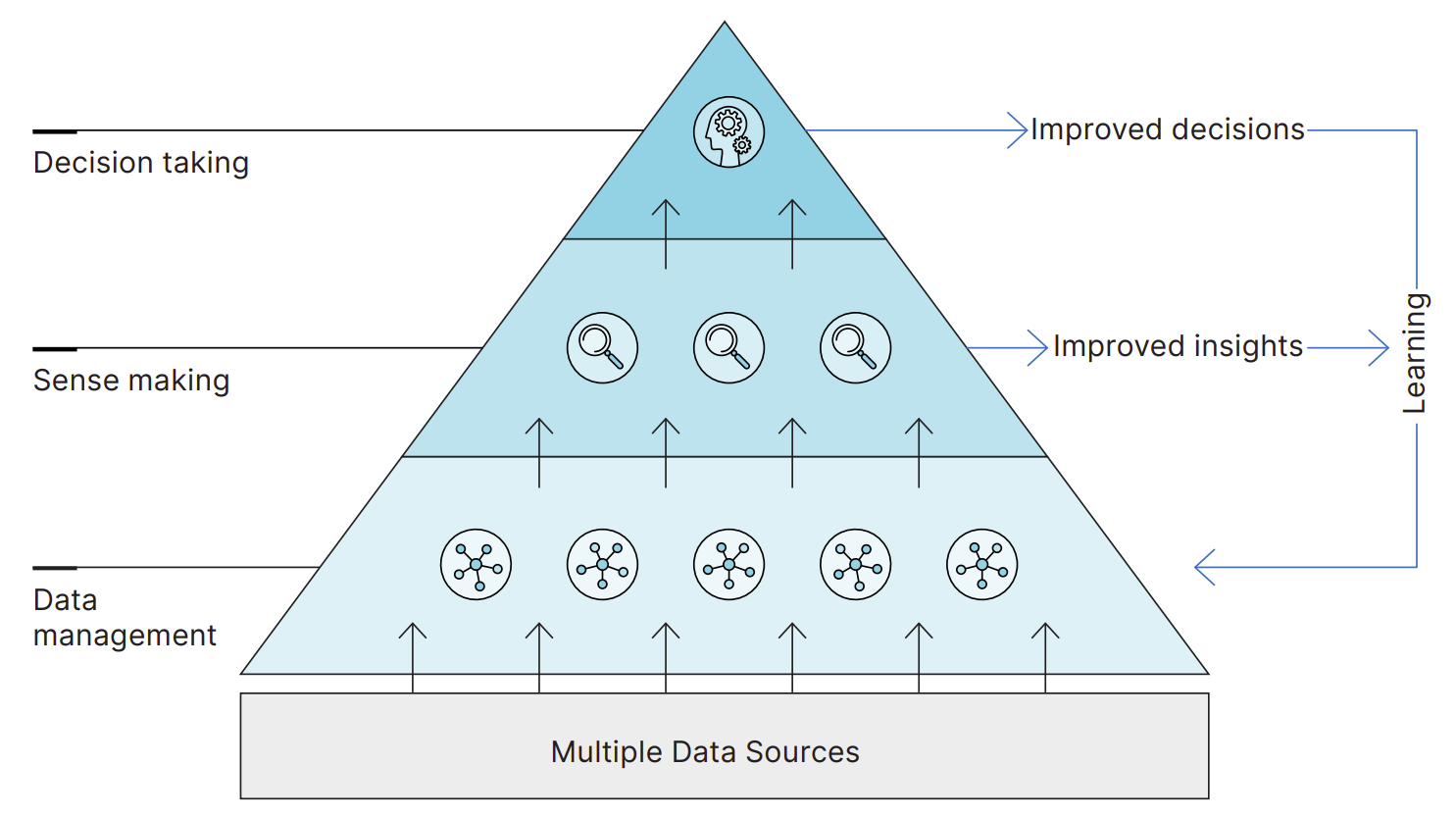
The Trial: Bromford Tunnel East Portal
In May 2022, a trial on DAARWIN was carried out at the western end of Bromford Tunnel East Portal. The trial was carried out in collaboration between Mott MacDonald and SAALG Geomechanics,(a Barcelona based start-up specialising in geotechnical digital tools) , supported by HS2 Innovation Team and Balfour Beatty Vinci JV. The primary aim of the trial was to better understand real world site conditions and ascertain whether DAARWIN can deliver efficiencies to enhance the implementation of the OM.
Description of the sset
Bromford Tunnel East Portal (BTEP) is located off Attleboro Lane in Water Orton, Birmingham. Figure 5 shows the layout plan of the site. The portal structure is approximately 33m (width) by 83m (length). The structure is formed by 1.2m thick diaphragm walls supported by one level of permanent concrete roof props at 88.55m APD and one level of temporary steel tubular props at 81.0m APD (Figure 6). The planned excavation depth varies from approximately 16m at the western end to 11m to the eastern end. The portal base slab will be cast in two stages to facilitate the launching of Tunnel Boring Machine.
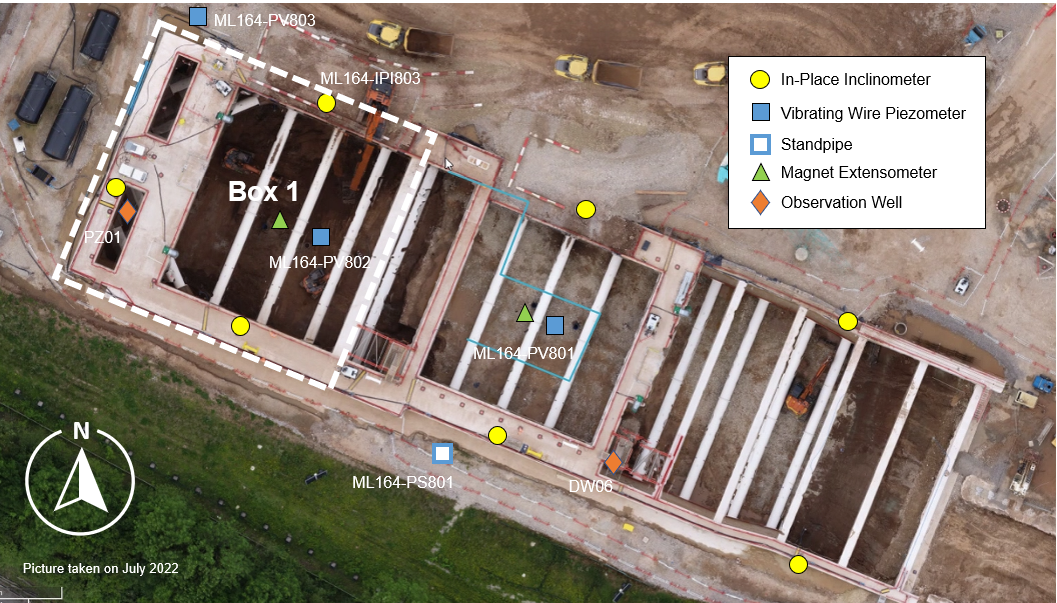
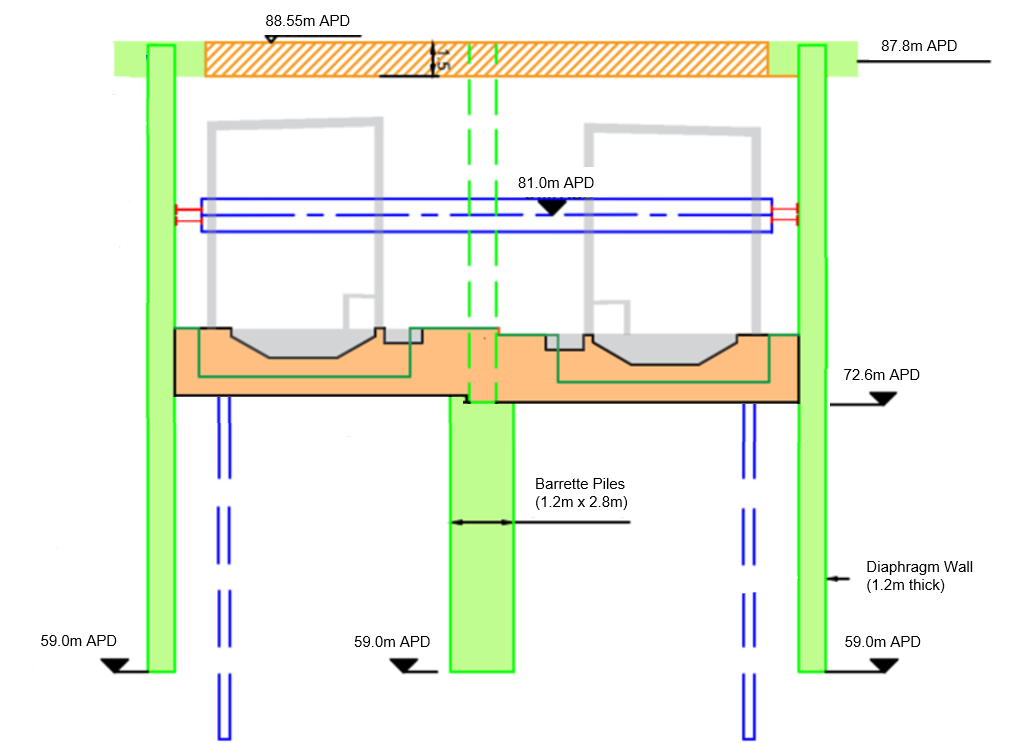
Ground conditions
The ground level at BTEP is approximately 88.5m APD. The ground conditions consist of about 4.5m glaciofluvial deposits, overlying the Mercia Mudstone group. Table 1 summarises typical descriptions of the encountered geology based on borehole data and Figure 7 shows a typical borehole core photograph for Mercia Mudstone Grade II/I in the vicinity of the BTEP site.
Table 1. Ground stratigraphy at Bromford Tunnel East Portal
Geology | Base Level (m APD) | Typical Description |
---|---|---|
Glaciofluvial Deposits, GFDUD-Co | 84.0 | Soft to firm brown slightly gravelly sandy CLAY. |
Mercia Mudstone, MM Grade IV | 81.0 | Firm to very stiff reddish brown, mottled greenish, grey, slightly and gravelly CLAY. Sand is fine to coarse. Gravel is subangular and sub-rounded. Fine to a medium Mudstone lithorelicts up to 25 mm. |
Mercia Mudstone, MM Grade III | 79.00 | Stiff to very stiff gravelly CLAY. Frequent presence of lithorelicts higher in size than 25 mm. This material is likely to be interbedded with extremely weak rock that has been softened and altered during the drilling process. |
Mercia Mudstone, MM Grade II/I | Not proven | Very weak to weak mudstone with subordinate siltstone and sandstone. Mudstones are generally structureless although some of the material does exhibit a ‘blocky’ structure. Interlaminated mudstones and siltstones occur within the formation. |
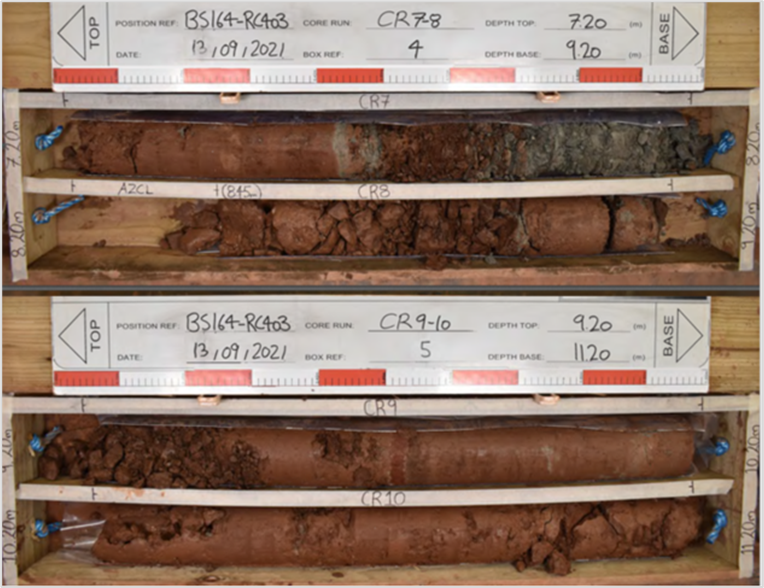
As described, the Mercia Mudstone Grade III is soil-like but its mass engineering behaviour is often closer to the behaviour between soil and rock.
Groundwater level is approximately 85m APD, 3.5m below ground level (Figure 8).
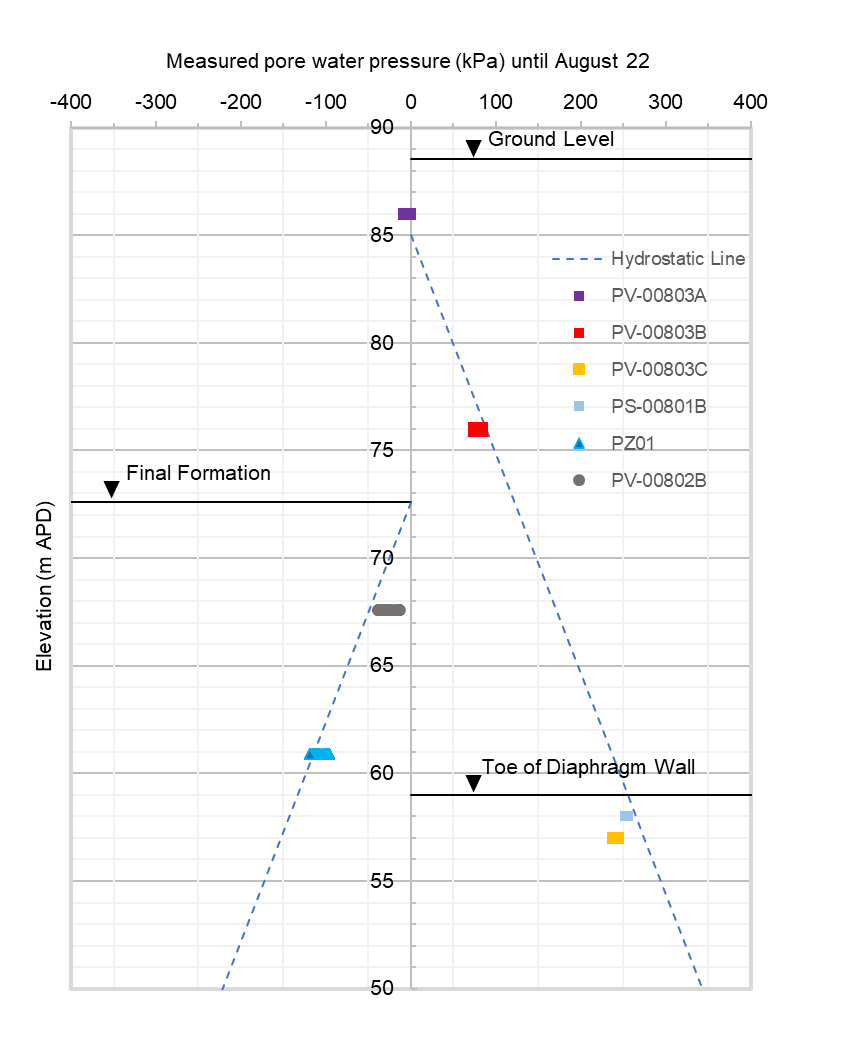
Instrumentation and monitoring
The primary instruments included seven in-place inclinometers (IPI) to monitor wall displacements. Empty inclinometer tubes were also installed adjacent to each inclinometer to provide redundancy in the monitoring scheme. To verify inclinometer readings and to increase confidence in the measured wall displacements, mini-prism survey points were installed. Other instruments included two magnetic extensometers, three fast response vibrating wire piezometers and one Casagrande standpipe as indicated in Figure 5.
Preparation for back-analysis
The excavation had commenced at the western end of the portal or known as Box 1 (Figure 5) for about four months when the DAARWIN trial started. The excavation depth was about 8m below ground level (Figure 9), which was the deepest at that time. Hence, Box 1 was selected for the trial to provide more valuable data for the back-analysis.
The readings from both automated and manually read instruments within Box 1 were collected, processed, and reviewed. The In-Place Inclinometer, ML164-IPI803 (Figure 5) was considered as the most suitable instrument to provide wall displacement data for the back-analysis. The as-built construction records and other site activities in the vicinity of the portal were gathered and reviewed. Figure 9 shows the progression of wall displacements against time alongside the excavation stages, using readings from the three sensors of inclinometer ML164-IPI803.
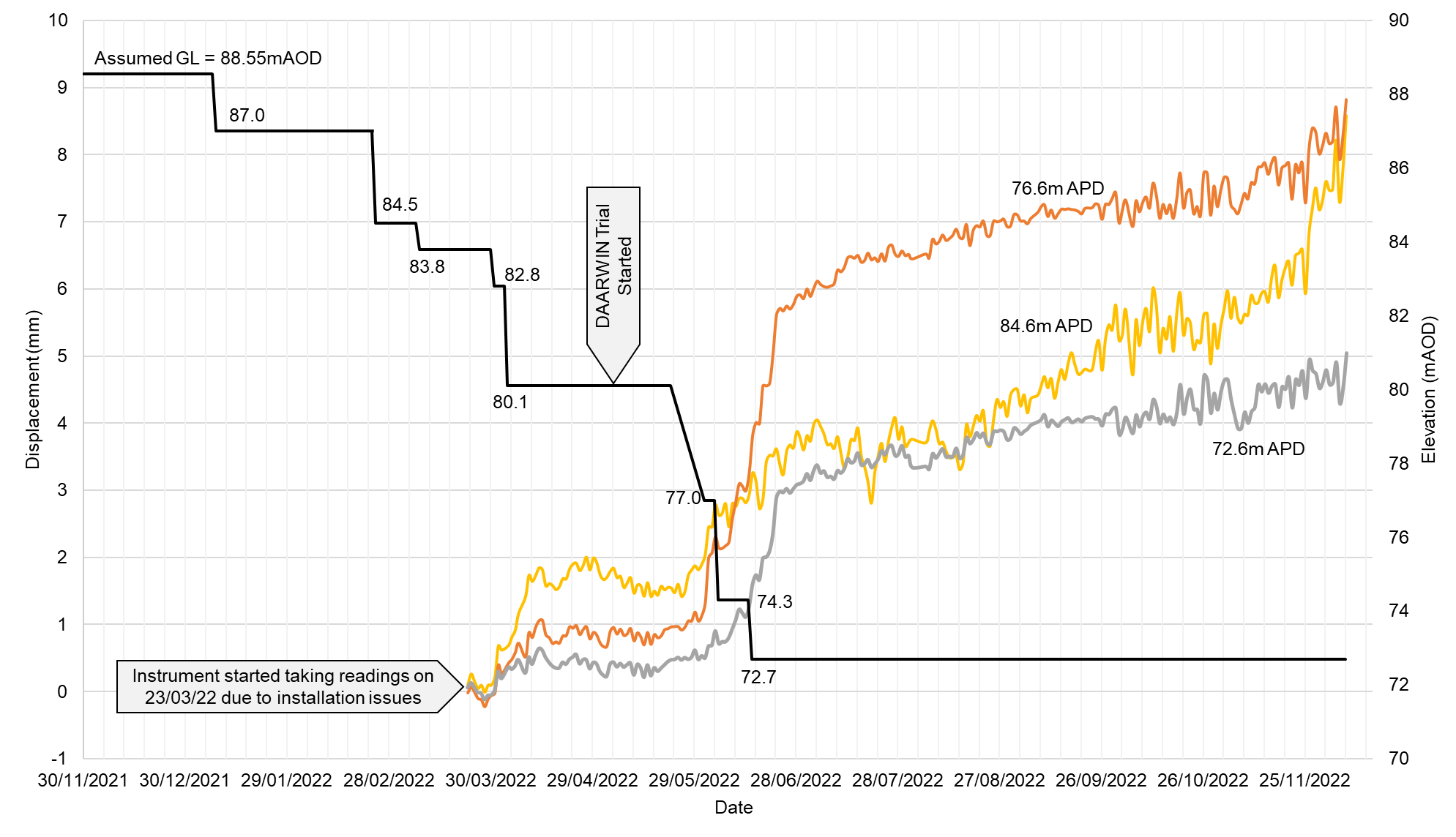
![]()
(a) Level 87.3m APD to 81.3m APD | ![]()
(b) Level 81.0m APD to 77.0m APD |
The observation of the exposed geology during bulk excavation had revealed significantly better rock mass characteristics than indicated by borehole logs (Figure 7). The observation on site indicated that the exposed mudstone is less weathered and fractured (Figure 10). This discrepancy is practically important as it indicated that the mass strength of the Mercia Mudstone Group is significantly higher than the strength values that were derived from the ground investigation. The higher fracture indices in Mercia Mudstone observed from the ground investigation is likely due to drilling-induced disturbance.
The dewatering activities, involving four abstraction wells within Box 1 and 6 additional wells at the remaining area of the portal, were effectively lowering the groundwater level inside the portal with a slight reduction in groundwater pressure near the toe level of the diaphragm walls (Figure 8).
Back-analysis using DAARWIN
The initial parametric studies indicated that the stiffness of the Mercia Mudstone Group is the most influential set of geotechnical parameters in influencing the displacement of the walls as compared to Mohr-Coulomb[12] strength parameters (i.e., effective cohesion, c’ and friction angle, f’). The DAARWIN back-analysis started with a design model that took into consideration as-built conditions including ground stratigraphy, groundwater profile, construction surcharges and structural member properties. The behaviour of all the materials was assumed to satisfy the Mohr-Coulomb failure criterion. The back-analysis was targeted on two excavation stages: 80.0m APD and 77.0m APD.
The DAARWIN back-analysis evaluated over 4920 numerical models (individuals) within 48 hours to determine the stiffness of the Mercia Mudstone Group (Table 2) using genetic algorithms. The algorithm started with an initial population of 151 individuals randomly generated until finding the solution after 50 generations and evaluating 4,920 numerical models. Out of all the models evaluated, 4,286 models were mimicking the measurements (4,286 good individuals out of 4,920 individuals evaluated). A snapshot of the DAARWIN back-analysis plot is shown in Figure 11. The individuals are filtered by prediction range – if the prediction from an individual is outside of a predefined “Buffer Zone”, this individual will be regarded as not valid (bad individual). The “Buffer Zone” is defined in relation to the reliability of monitoring data. Due to the reality of instrumentation installation, calibration, measurement, and data processing, it is inevitable that monitoring data includes a level of error, either as random or correctable errors. Given the relative smaller magnitude of measured displacements (Figure 9 and Figure 13), the “Buffer Zone” was defined as +/- 0.5mm.
Table 2. Back-analysed stiffness values for Mercia Mudstone (MPa)
Soil/Rock Stiffness | Model input1 | Optimal Mean2 | Min2 | Max2 | Best Individual3 | Best Estimate4 |
---|---|---|---|---|---|---|
MM-IV_E’ | 82 | 129 | 71.6 | 186 | 75 | 62 |
MM-IV_∆E’ | 47.7 | 52.5 | 18 | 87 | 20 | 34 |
MM-III_E’ | 225 | 252 | 215 | 288.7 | 270 | 164 |
MM-III_∆E’ | 72.5 | 166.4 | 154.5 | 178.3 | 195 | 42 |
MM-II_E’ | 430 | 334 | 288 | 380 | 335 | 308 |
MM-II_∆E’ | 59.5 | 36.4 | 5.0 | 67.8 | 10 | 39 |
MM-I_E’ | 669 | 604 | 520 | 687 | 710 | 464 |
MM-I_∆E’ | 70 | 77.4 | 51.8 | 103 | 80 | 41 |
Note: 1. Model input – manually input parameters for the commencement of back-analysis; 2. “Optimal Mean” – average of back-analysed parameters within the “buffer zone”; “Min”, “Max” – Optimal Mean +/- Standard Deviation; 3. “Best Individual” – best combination set of parameters that give predictions with the least discrepancy to target measurements, about 75% within “Buffer Zone”; 4. “Best Estimate” – parameters derived from engineering judgement for the use of the Observational Method.
Based on the back-analysis results, “Optimal Mean” values including their Standard Deviation and “Best Individual” values were determined by DAARWIN. Engineering judgement was then applied to derive a cautious set of “Best Estimate” values, which can be used for the application of the OM. The back-calculated stiffness values for Mercia Mudstone were summarised in Table 2 and presented graphically in Figure 12 . Figure 13 compares the wall displacement predictions against measurements based on inclinometer ML164-IPI803. The maximum wall displacement at Box 1 was less than 10mm, which was significantly lower than the pre-defined wall trigger limits, i.e., Red Limit of 40mm. The wall trigger limits were governed primarily by the structural crack width criterion for the retaining walls (i.e., serviceability limit state criterion) and, to some extent, by the presence of an Esso pipeline on the south side of the portal.
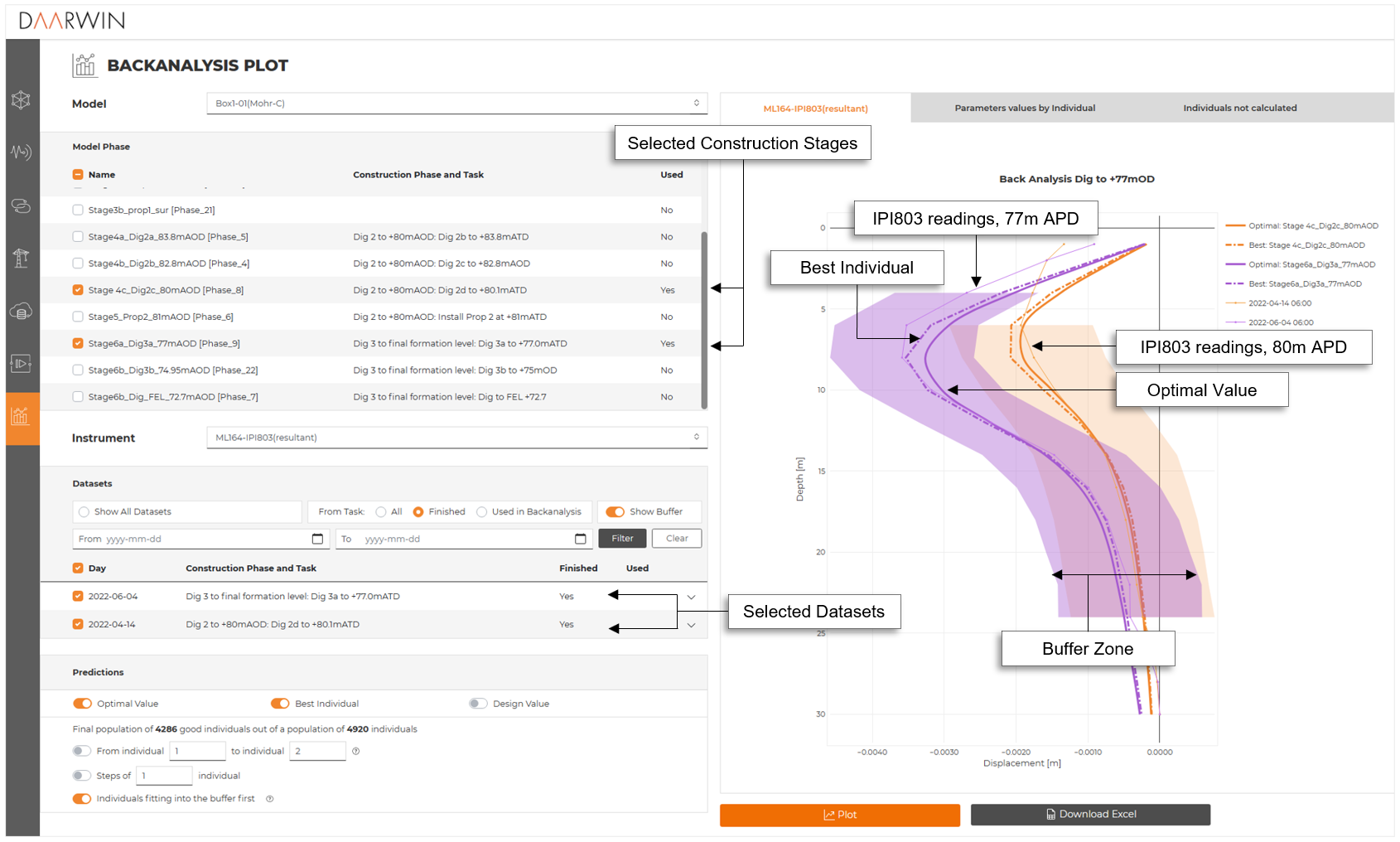
Outcomes
The DAARWIN trial was completed in August 2022. The trial revealed:
- high mass strength and mobilised ground stiffness of the Mercia Mudstone.
- an effective dewatering regime during the box excavation at BTEP.
- measured wall displacements at BTEP Box 1 were relatively small, less than 8mm as compared to wall displacement “Red Limit” of 40mm.
These findings indicate a high probability that the temporary steel props at BTEP Box 1 could be omitted. It also revealed opportunities to eliminate heavy and constricting temporary works for excavation projects using similar construction methodology located in similar geological conditions, provided the OM is used effectively.
The trial has illustrated DAARWIN can enhance the implementation of OM as a near real time back-analysis tool and as an integrated platform for hosting the combined project database including design information, monitoring data and construction activity details. It indicates the monitoring data can be easily stored, visualised, and correlated with the corresponding construction phases, enabling a thorough review of the monitoring data against construction activities and predictions. This facilitates the back-analysis which is often complex and time consuming, allowing the use of OM on much larger scale applications like HS2 than was possible using conventional methods to maximise OM benefits.
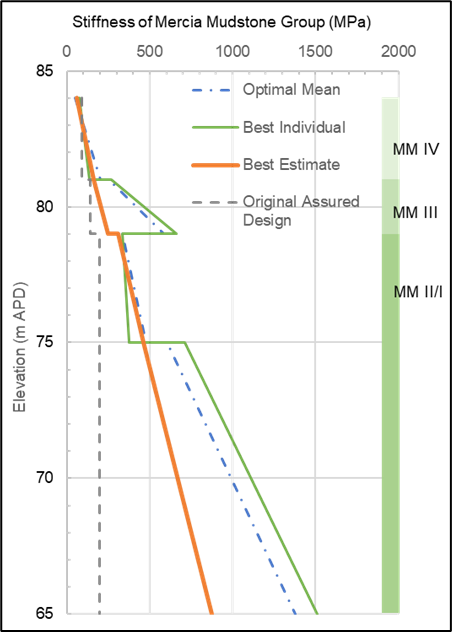
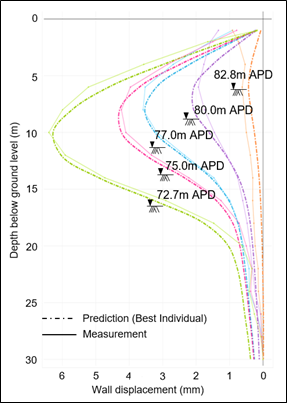
Learnings and recommendations
The DAARWIN trial was considered highly successful. It had tested DAARWIN as a new technology employing machine learning to enhance the OM. The key findings and lessons learned from the trial had been shared with HS2 and Balfour Beatty Vinci Joint Venture through the Innovation Knowledge Share workshop. The OM, employing DAARWIN had also been applied at the eastern end of BTEP to omit the temporary steel props and recommended to be used for the adjacent portal structure and retained cut.
The trial had also identified further opportunities and areas for improvement that can help to improve the use of DAARWIN to maximise the benefits of OM. They are:
- DAARWIN as a digital twin – In addition to enhancing the OM, the trial indicates the potential of using the DAARWIN platform as a digital twin for a project. Its ability to connect engineering design and as-built performance can provide a coherent and informed basis for future asset management. For example, it can support the HS2 operational phase for more effective and efficient long-term maintenance of their assets. It can also provide greater transparency and traceability during the implementation of OM, which is important for project stakeholders.
- Instrumentation and monitoring (I&M) – it is fundamentally important to have a well-designed I&M system in OM, focusing on quality not quantity. The purpose of each type of instruments should be clear, either primary or secondary system. The design of the primary system should be robust with redundancy in the system. The primary instruments for the DAARWIN trial at BTEP were in-place inclinometers (IPIs). Directly adjacent to the IPIs were spare reservation tubes with ABS (Acrylonitrile-Butadiene-Styrene) inclinometer casings installed and baselined with a manual probe. It was evident from the trial that the spare tubes proved to be very useful when an IPI indicated anomalous readings. Mini prisms were also provided at BTEP to provide additional insight of wall behaviour and to cross-check the IPIs. However, the manually read mini prisms data at BTEP were often difficult to resolve with confidence. It is recommended that an autonomous optical monitoring system using Automated Total Stations (ATS) should be used to record the relative positions of the prisms if absolute positions are less reliable. Wall convergence monitoring using automatic optical system (ODS) should also be provided. The ODS could replace the IPIs as the primary system once the excavation reaches the level where the wall convergence monitoring sensors are installed. The wall convergence monitoring, if correctly implemented, can provide a direct measurement of wall behaviour, requiring minimum interpretation and enabling rapid decision making during the implementation of OM.
- Collaborative working – it is essential for the contractor and designer to work as a single team, i.e., no “them/they” but “we/us” to ensure the success of OM. The OM is not a traditional design. It does not follow a traditional model which is predominantly linear with very limited or no feedback loops. In OM, both design and construction requirements need to be matched and implemented rigorously. To achieve this, close teamwork and mutual trust are key. It is recommended that the OM designer to have regular face-to-face workshops with the contractor to create a truly effective and collaborative team. During the trial, the designer and the contractor had only had one on-line meeting every week. It is recommended that the frequency of the meetings should be increased to at least three times every week during the implementation of OM to improve information sharing and to ensure OM rules are adhered to, e.g., timely and effective implementation of contingency measures if required. The trial also indicates on-site presence by the OM designer is essential to gather daily site information for the back-analysis and to ensure effective two-way communication between the OM designer and construction team.
- Roles and responsibility – clarity is essential during the implementation of OM. The trial indicates a single point of contact from the OM designer and construction team is essential. The OM designer lead should have full authority and responsibility to implement the design requirements for OM implementation, whilst the site control lead should have the full authority and responsibility to implement the OM construction requirements. This ensures critical observations can be obtained reliably and in a timely way, enabling timely implementation of pre-planned contingency measures.
- Understanding of Mercia Mudstone – the trial has provided a better understanding of the characteristics of Mercia Mudstone in terms of in-situ strength and stiffness in retained excavations, which will offer wider benefits for HS2 and future projects in the region given the extensive distribution of the Mercia Mudstone.
Conclusion
The Observational Method, when used effectively, can deliver:
- major cost, time, and carbon savings.
- enhanced safety by eliminating heavy and constricting temporary works, focusing on the importance of teamwork, good communication, clear procedures, construction control and pre-planned contingency measures.
- more opportunities for driving innovation, re-using back-analysis outputs in future design and reducing over-conservatism in geotechnics, creating more value to asset owners to achieve better outcomes.
The new technology, DAARWIN based on machine learning algorithms can enhance the implementation of OM as a near real time back-analysis tool and as an integrated platform for hosting the combined project database including design information, monitoring data and construction activity details. The efficiency created by DAARWIN enables the use of OM on much larger scale applications like HS2 than was possible using conventional methods to maximise OM benefits. In addition, it potentially can be used as a digital twin for a project, providing a coherent and informed basis for more effective and efficient maintenance of assets.
Acknowledgements
The authors would like to thank colleagues at Mott MacDonald (MM) and SAALG Geomechanics, in particular Imran Farooq (MM), Rob Talby (MM), Christopher Brook (MM), Michael Vance (MM), Sophie Smith (MM), Jules Birks (MM), Ben Marriott (MM), Zhandos Orazalin (MM), Miguel Mucientes Rufo (MM), Tim Hou (MM), Markosz Topalidisz (MM), Ray Larkins (MM), Sebastian Drew (MM), Joe Mazgajczyk (MM), Echo Ouyang (Ex-MM), Ying Chen (Ex-MM), Ignasi Aliguer (SAALG), Carlos Acosta (SAALG), Claudia Cano (SAALG), Maria Santaeularia (SAALG) and Toni Baraut (SAALG).
As well as the members of HS2 and BBV that helped us to carry out this Innovation STRATEGIC Project, in particular Lyndon Trinder (BBV), Chebli Matta (BBV), Stefan Bernhard (BBV), Tom Nolan (BBV), Nick Sartain (HS2), Jonathan Kelly (HS2) and Charlotte Hills (HS2).
References
- DAARWIN cloud-based platform . Accessed on 28 July 2023
- Horizon’s Challenge 01”: European Commission, Directorate-General for Research and Innovation, Horizon Europe: Strategic Plan 2021-2024, Publications Office of the European Union, 2021.
- BS EN 1997-1:2004, Eurocode 7 – Geotechnical design, Part 1: General rules, London: British Standards Institution.
- Technical Report: Implementation of SAALG’s Monitoring Data Analysis Technology (Platform DAARWIN) on High Speed 2, Contracts C2 & C3, United Kingdom – Boddington Trial (SAALG Real-Time Back-analysis, 1MC07-CEK-PM-PLN-C003-000011).
- Powderham A J. The observational method – learning from projects. Proceedings of the Institution of Civil Engineers, Geotechnical Engineering. 2002; 155 (1): 59–69.
- Powderham AJ, O’Brien A S. The Observational Method in Civil Engineering, minimising risk, maximising economy. CRC Press, 346 pp. 2021.
- Nicholson D, Tse C M, Penny C. The observational method in ground engineering: principles and applications. CIRIA Report 185. 1999.
- Liew H L, Farooq I, Place D, Steele B and Vitorino S. An innovative verification process speeds construction of Crossrail’s Moorgate shaft. Crossrail Project Infrastructure design and construction. 2016; Volume 3: 461-474.
- O’Brien A S, Liew H L. Delivering added value via advanced ground investigations for deep shaft design in urban areas. D.F.I. Conference, Rome. 2018.
- O’Brien A S, Chen Y, Liew H L. Creating value through Observational Control. 11th International Symposium, Field Monitoring in Geomechanics. 2022.
- De Santos C. Backanalysis Methodology Based on Multiple Optimization Techniques for Geotechnical Problems. Ph.D. thesis. Universitat Politècnica de Catalunya – BarcelonaTECH. 2015.
- Coulomb, C. A. (1776). Essai sur une application des regles des maximis et minimis a quelquels problemesde statique relatifs, a la architecture. Mem. Acad. Roy. Div. Sav., vol. 7, pp. 343–387.
Peer review
- James Daniel HS2 Ltd